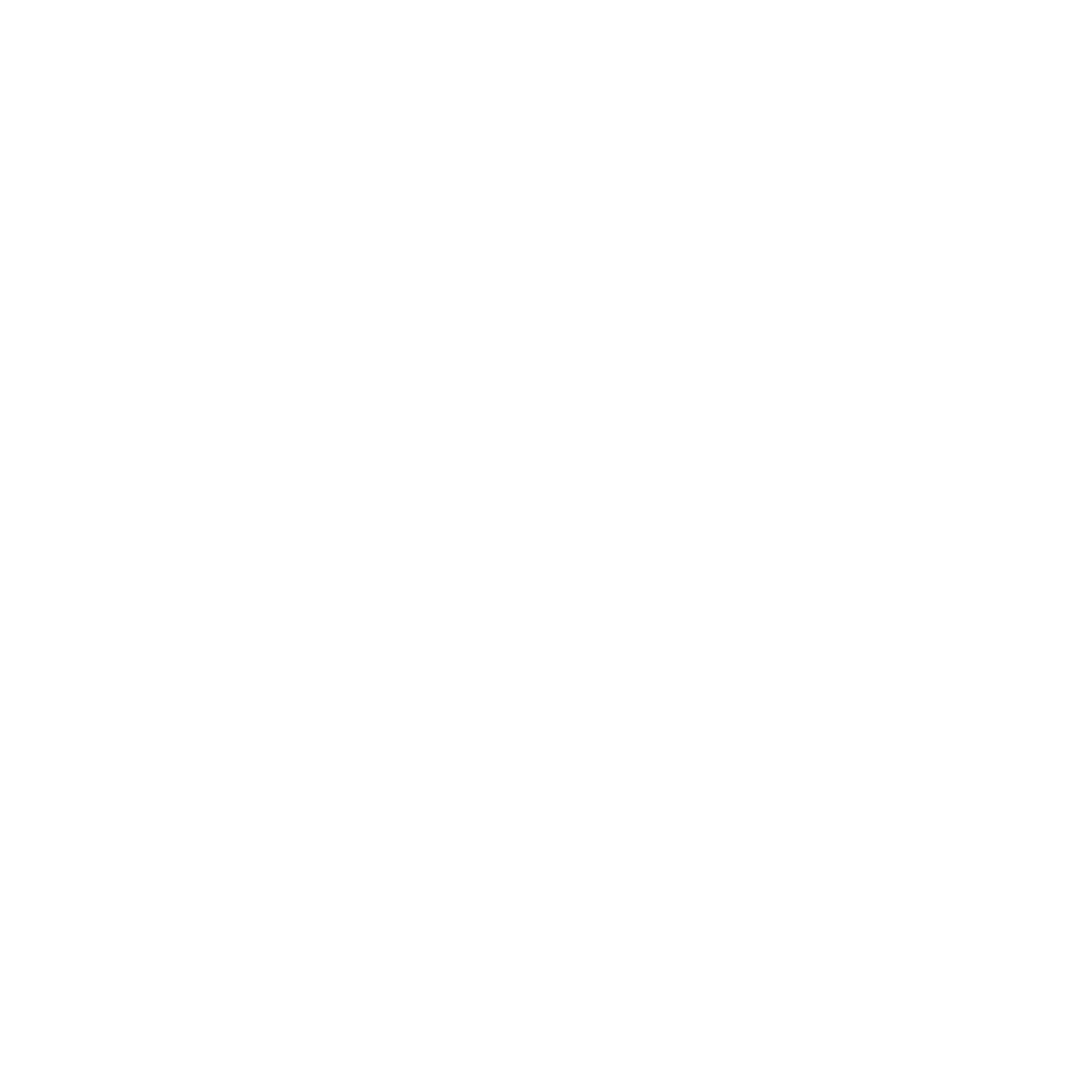
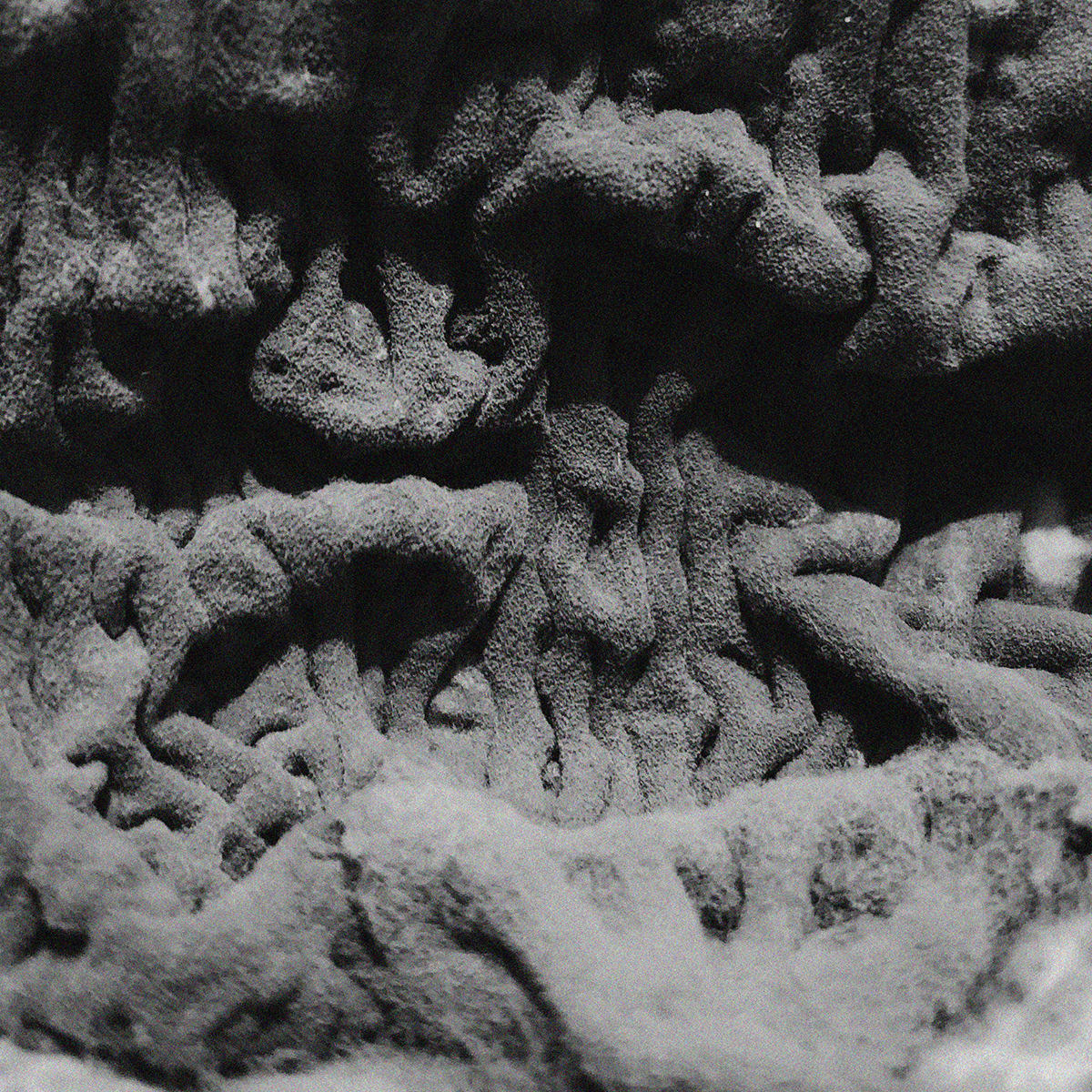
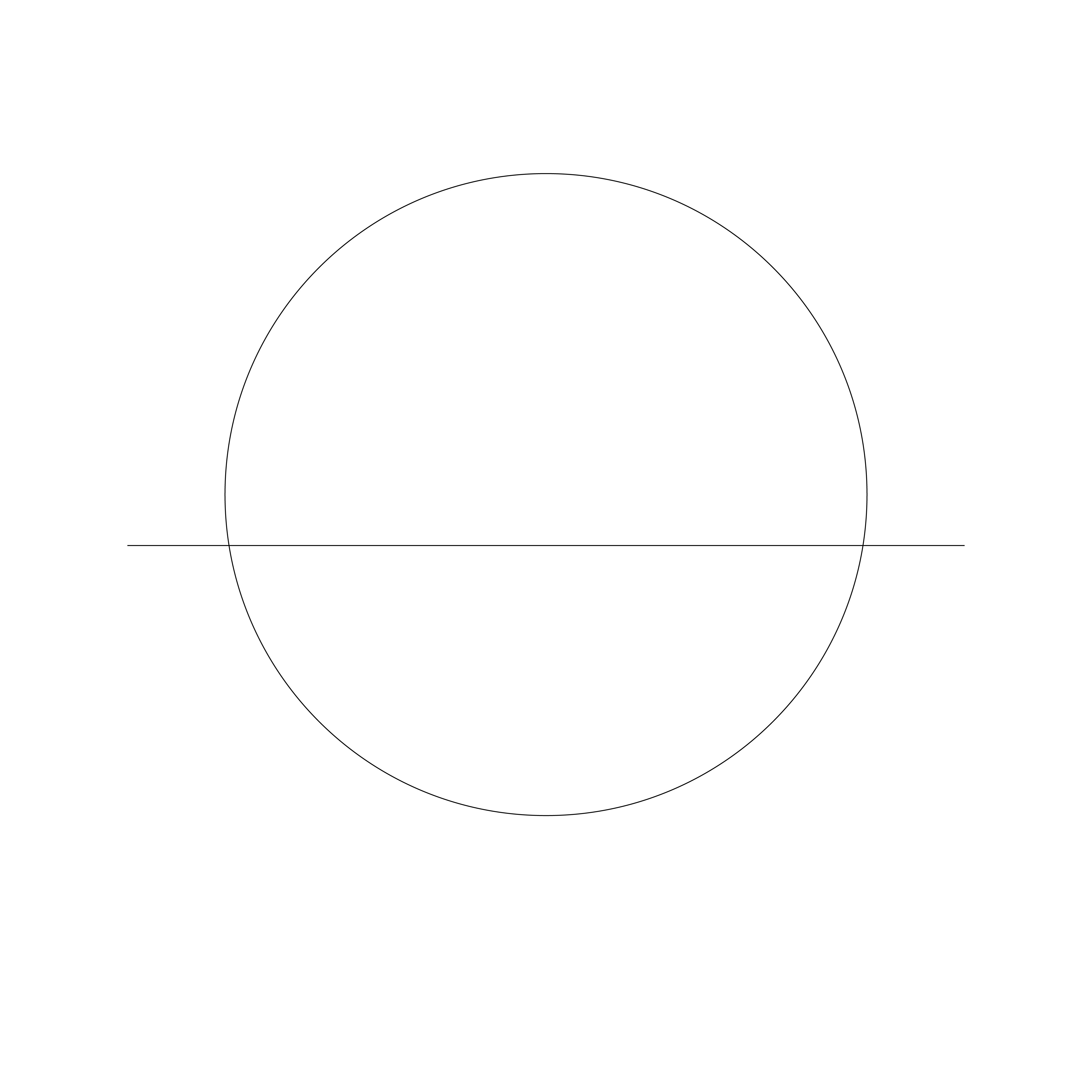
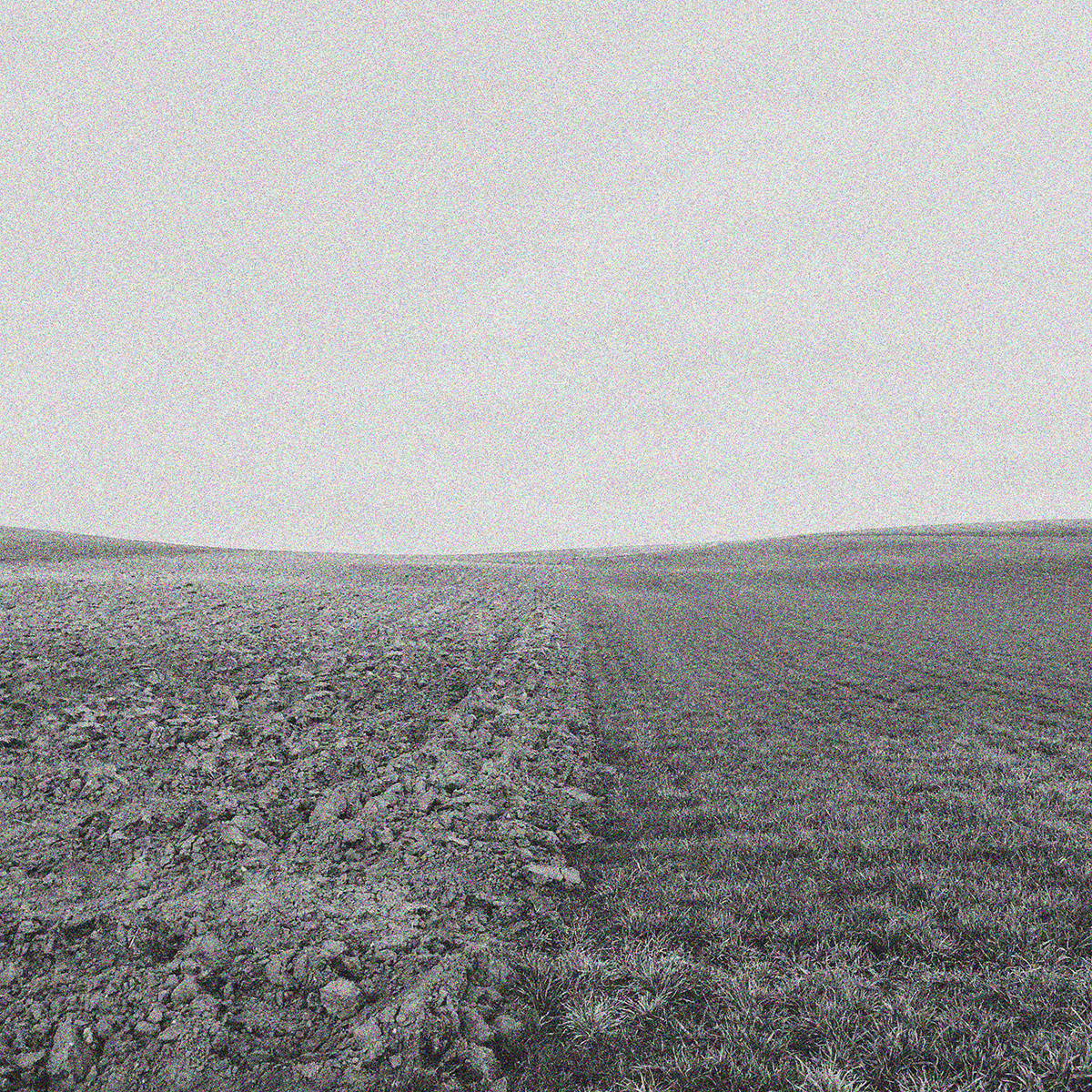
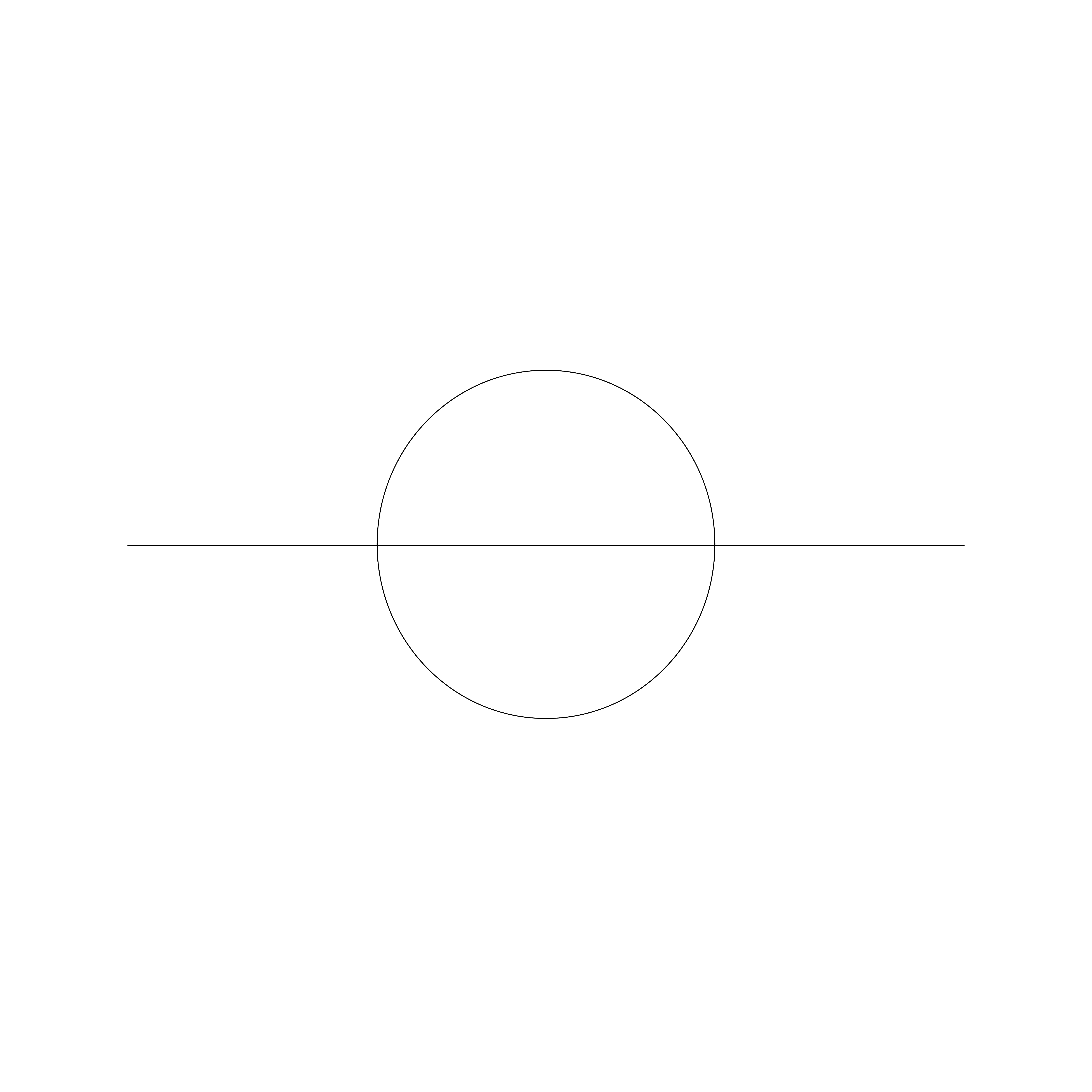
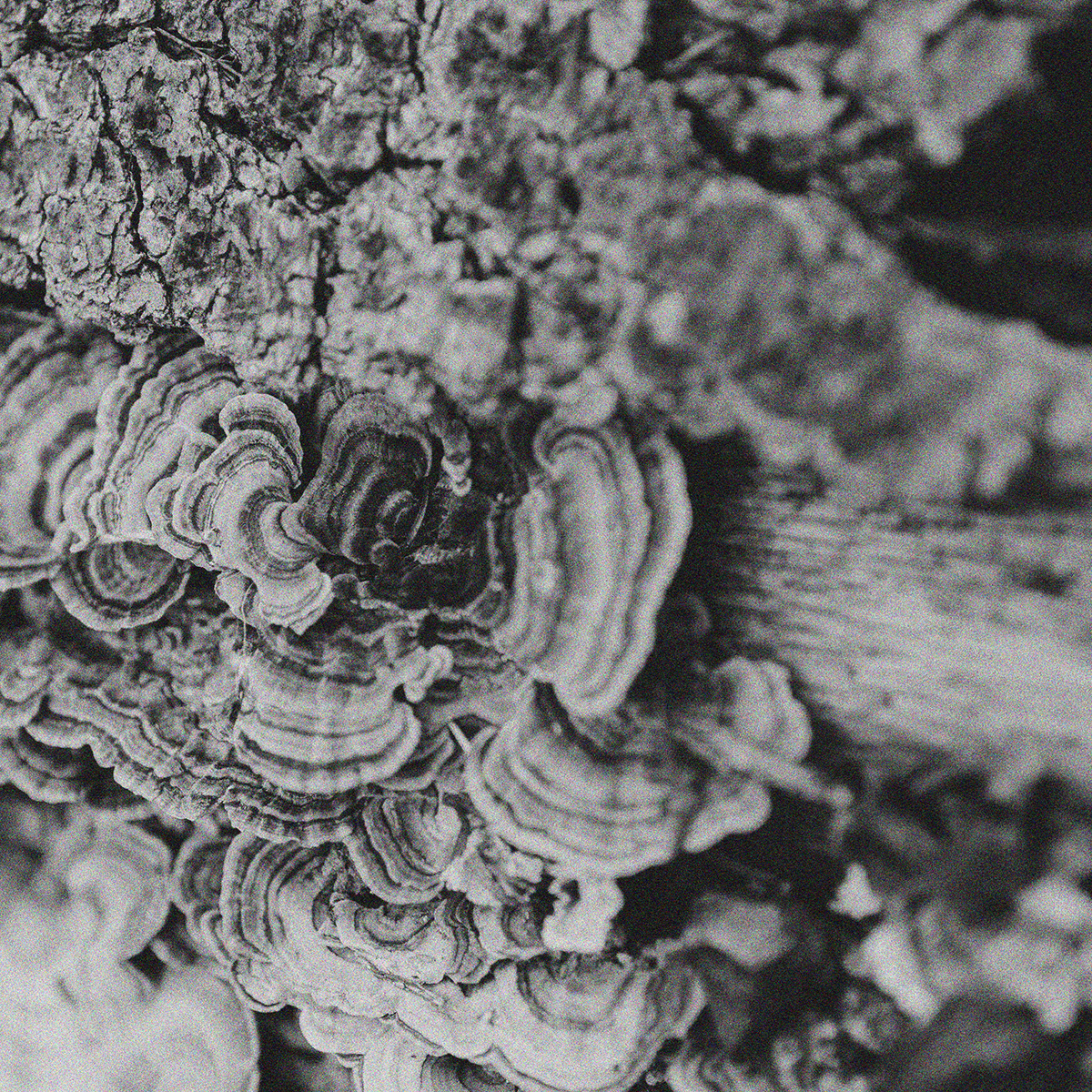
Scale
Perhaps now, more than ever, it’s important to be thinking about scale. As the story goes, humans, who for centuries have disavowed their relation to the planet, will never again have the privilege of forgetting it.[1] In short: Biological systems have become geological forces. The timescales of human history intersect with geological history to such an extent that the former may one day soon be history.[2] This observation, which is increasingly commonplace across the sciences, arts, and humanities, places an existential urgency on “scalar thinking,” or the “ability to move across spatial and temporal scales.”[3] If Homo sapiens is responsible for this collision of spatial and temporal scales, then it’s a collision that has never been experienced before, since it puts its own existence at risk.
Geobiologists and scientists working in adjacent fields map these interscalar relations, focusing specifically on the coevolution of life and Earth, and by doing so are able to bring the complex, nonlinear relations between the biosphere and geosphere into view. According to the American Academy of Microbiology, the emergent field of geobiology is making incredible progress toward “understanding Earth’s history, including the origin of life and the evolution of global biogeochemical cycles”; “improving our knowledge of how cell-to-cell communications and microbial community interactions can control global biogeochemical processes”; and “producing useful substances in easier and cleaner ways, as compared with conventional engineering approaches.”[4]
Still, if geobiology is itself a relatively new interdisciplinary science (officially founded in 2003 but coined in the 1930s), then it has a slightly more idiosyncratic genealogy that can be traced to the Soviet biogeochemist Vladimir Verdansky (1863–1945), who popularized the concept of the noösphere (a combination of mind [νόος] and sphere [σφαῖρα]). Briefly, the noösphere was hypothesized to be the next layer of geological evolution (although it’s debatable whether it was the French Jesuit Pierre Teilhard de Chardin or Édouard Le Roy who actually invented the term). If the biosphere was the last major step in planetary development, after the geosphere, then the noösphere was thought to be a geological formation that takes shape in the wake of the biospheric development of cognition—in short: Our species has a geological footprint insofar as it prepares the way for the emergence of a thinking planet that transcends individual minds that make it possible.[5]
Despite the fact that Verdansky’s insights about the next stage of evolution are far from the data-driven work of geobiologists, paleobiologists, and biogeochemists today, they still haunt their practices in strange ways: Scientific thought about planetary complexity (of the sort geobiologists deal with) relies on information processing that exceeds the components that make them possible. Their work depends on computing systems that turn vast quantities of data about diverse scales of geological and biological activity into complex mathematical models that trace causal patterns and probability distributions. In this way, theories of geobiological complexity in mainstream science are mediated by the computational sciences, mainly because algorithms running powerful computers are able to integrate across vastly different scales of biological and geological organization. In recent years, this reliance on data science to do the experimental and theoretical work of “Science” has led to no shortage of criticism among concerned scientists, leaving many to worry that scientific thought is now in the hands of machines.[6] Science, on this template, is purely formal operation, in principle no different from the pure mathematical formalism of David Hilbert (early twentieth century), who postulated that all mathematical axioms could be proven by a finite set of mechanical operations. The implication here is that the relations between the biosphere and the geosphere are mediated by computing systems operating at a planetary scale—a sort of planetary-scale formalism that becomes the computational equivalent of the noösphere.
While this last statement should serve as a kind of provocation and should not be confused with the position I’m endorsing, some version of it has already been outlined by Jennifer Gabrys[7] and Benjamin Bratton.[8] In different ways, they affirm that there is no planet without computational technologies. Whether it’s the “Blue Marble” or the “Anthropocene,” we have no conception of the planetary, and the impact our species has on it qua geological force, without computing. It’s the data gathered from a myriad of tools—remote sensing technologies, surveillance equipment, pollution-monitoring mechanisms, etc.—and processed by large-scale mathematical models that give us our current notion of the “planetary.” The existential urgency to think and intervene on the planetary scale is in some fundamental sense an artifact of computing—or in other words: it’s by computational design. Indeed, our modern, scientific conception of anthropogenic climate change is a product of data science.
From this extensive work in the geological and atmospheric sciences, plants have emerged as essential “geobiological agents.”[9]Paleobotanists, for instance, have shown that the first plants developed as organisms that lacked vascular tissues and were restricted to wetlands, and real diversification only occurred with the development of vascular tissues. From there, the evolution of roots redesigned landscapes, and the development of leaves and photosynthesis impacted the carbon cycle, and so on. Terrestrial vegetation of various sorts has been shown to shape geological and atmospheric phenomena at both short and long timescales: On the one hand, they affect how land surfaces affect “atmospheric greenhouse gasses, and their precursors” within the timescales of minutes and years; and on the other hand, “geobiological actions are expressed by their accumulated influence on the organic and inorganic carbon cycles” over millions of years (189).[10] And within the applied sciences, the phytoremediation capabilities of certain plant species, e.g., Thlaspi caerulescens, serve as an important reminder that plants are reparative geological architects.
It’s precisely the geobiological agency of plants, or in other words, their ability to link vastly different scales of organization, that have made the computational tools of mathematical physics so attractive. There’s a much longer story to be told here about the rise of computational complexity in the biosciences, and more specifically, about the importation of mathematics into biology, but suffice it to say that integrating different scales of plant organization (molecular, cellular, multicellular, interorganismic, and ecosystemic) increasingly depends on the computational sciences to reveal the complexity of interacting spatial and temporal scales.[11] Admittedly, the plant sciences have been slower to incorporate mathematical models into their work—save their use in genomics (expression and regulatory networks), morphology, and physiology[12]—than other areas of the biosciences; however, it’s become increasingly important to use mathematical models to study the interaction between, for example, root and soil systems (“root-soil models”[13]) as well as how terrestrial vegetation affects the carbon balance.[14]
Piecing these data together with fossil records from the earth’s first ecosystems in order to predict future events gives us a rough sketch of how the geobiological agency of plants is configured today. But this kind of science is only possible using what the reviewers for the American Academy of Microbiology call a “fantasy computer”: a computer capable of synthesizing data from scales of activity that seem to have little to no causal relation to what traditional experimental practices would be capable of synthesizing. Still, we’re told that via machine learning and other forms of AI-driven research, patterns of activity and nonlinear causal regimes begin to emerge. This is the future of geobiology.[15]
This, of course, is a far cry from the story we’re told about the importance of scale and plants in much of the plant studies literature. If there’s a “plant turn” underway in the posthumanist and ecocritical circles of the humanities, then it’s not with an eye to understanding the computational artifice of earth systems and causally integrating various (geo, bio, social) scales of activity. It’s decidedly in order to “make kin” and establish an interspecies symbiosis[16] that plants have become the new “animals.”[17] By using noninstrumental, nonrational, and nonhierarchical modes of relating to plants—in the interstices of mainstream science—they have emerged as “teachers” and “collaborators” able to reconnect humans to the agency of the earth and its nonhuman inhabitants. And it’s this nonhierarchical connectivity with scales of planetary activity that plants (and hence critical plant studies) play a key role in mediating the ecological crisis.
If there’s a nod to the information sciences and the history of cybernetics in plant studies literature, then it’s generally metaphorical and used to illuminate how plants signal, have elaborate communication networks, and may even have a kind of “neurobiology.”[18]But this work, especially under the umbrella of critical plant studies, is done in the shadow of mainstream science and certainly not with the ambitions of saying the planet is actually a computer.
And yet, there’s a version of the argument in favor of planetary artifice (or even the computational planet) that makes good sense and moreover can help us think scale in a way that is appropriate to plant biology. The planetary is an abstraction, and so is the notion of ecological crisis, the loss of biodiversity, and so on. Laboratory experimentation is important for generating these conclusions. But to make reliable claims about global warming, for example, experimental evidence is fed into mathematical models that run on computers. Computations are performed to generate probabilities, and models of planetary warming are produced. Ultimately, the urgency to think and feel at the planetary scale is mediated by computer models. In this context, the planetary is an incredibly useful abstraction, but as Alfred North Whitehead would say: We have to take care to not overestimate what such an abstraction can be used to explain.[19]
Let’s take the use of Paulownia trees in the environmental sciences as a way of framing this. Formally, a genus with seventeen species of hardwood trees native to East Asia, these trees are considered to be an “environmental powerhouse” because of their ability to capture carbon.[20] This in turn impacts greenhouse concentrations in the atmosphere and the earth’s ability to cool. Not only this, but Paulownia trees thrive in degraded lands and help to improve soil health,[21]which makes them perfect for wider ecosystem restoration projects—at once able to capture atmospheric carbon and restore soil health. Paulownia trees thus have a remarkable ability to connect various scales of planetary activity. And yet to make sense of Paulownias’ activities, and fit them into the wider frame of sustainably mitigating C02,[22] requires tools from a host of interdisciplinary science fields.
Scientists get into trouble, however, when they start thinking that biological scales of spatial and temporal organization are reducible or explainable in terms of other scales of organization—as if one follows from the other. This is not to say that one scale of organization (biological) is completely external to another (physiochemical), or that the one does not incorporate the latter in some way. Nor is it to say that large-scale models don’t give us a good sense of emergent patterns over time. The case of the Paulownia trees makes this explicit. Rather, I want to argue that when it comes to pinpointing the causal mechanisms operative in the evolution and development of biological systems—whether a unicellular system or a multicellular organism, like a plant—then we need conceptual frames that appreciate how the spatial and temporal scales of biological activity matter: They represent a scale of earthly activity irreducible to physicochemical scales of activity. But why?
The fact is: The tools of mathematical physics used to explain the organization of matter are not sufficient for explaining the organization of biological matter. This is because nonlinear dynamical systems require predetermining the phase space of possible trajectories, and biological systems determine their own phase spaces as they develop.[23] What biologists are beginning to realize is that there is no way to predetermine how an environment will affect the organism’s onto- and phylogenesis. It means something different each time, and as such, there’s no mathematical ability to predetermine the phase space of possible change. This is determined in and through the development of the phenotype. Consequently, there’s no external marker (genetic or epigenetic) that would allow us to trace causal mechanisms responsible for phenotypic development—if you have x, then you’ll gety.[24] It’s a different environment for every system. This is fundamentally at odds with complex systems modeling in the biosciences and certainly has no place in the large-scale climate models that map the causal patterns of earth systems.
This research in theoretical biology has massive implications: There are scales of material organization that can’t be causally integrated with others. At a certain level of material organization phenomena emerge that can no longer be explained solely in terms of the previous level of organization, which means that new methods of research are required to investigate these phenomena. As the Russian biologist Boris Zavadovsky wrote in 1931:
The true task of scientific research, is not the violent identification of the biological and the physical, but the discovery of the qualitatively specific controlling principles which characterize the main features of every phenomenon, and the finding of methods of research appropriate to the phenomena studied. [ . . . ] But biological laws do not in the least lose thereby their material quality and cognisability, requiring only in each case methods of investigation appropriate to the phenomena studied.[25]
Perhaps this is where a critical and speculative discourse on plants is needed most: We need to affirm that plants incorporate and shape various spatial and temporal scales of activity (geological, atmospheric, etc.), and that they do so in ways that tend to have this or that effect (which models detect). However, the way in which they relate resists being captured by finite schemes of description. This means that their manner of relation, in this instance, cannot be externalized in an objective model. What we lack is a conceptual and mathematical vocabulary for the genotype-epigenotype-phenotype ensemble we call the “plant,” which clearly connects radically different scales of temporal and spatial organization but which resists being reduced to any of them.
Whitehead often speaks of the dipolarity of experience (an occasion is at once subjective and objective, interior and exterior, subject and superject), and it might be worth returning to this notion in order to tackle how the plant at once relates and doesn’t relate to different scales of organization. This conceptual scheme shouldn’t be to the exclusion of computational models for mapping general tendencies. Instead, we should put them to the pragmatic test: Do they help isolate areas for intervention or not, spaces where biology and geology overlap in important ways? We just can’t mistake these abstractions for concrete explanations of biological causality. Which is precisely what the geobiologists, climate scientists, and technofetishists in postcontinental philosophy do. Instead, we need abstractions that resist the temptation to explain away what fundamentally resists being reduced to a causal explanation.
Notes
[1] Isabelle Stengers, In Catastrophic Times: Resisting the Coming Barbarism, trans. Andrew Goffey (Open Humanities Press, 2015).
[2] Dipesh Chakrabarty, The Climate of History in a Planetary Age (University of Chicago Press, 2021).
[3] Gabrielle Hecht, “Interscalar Vehicles for an African Anthropocene: On Waste, Temporality, and Violence,” Cultural Anthropology 33, no. 1 (2018): 109–41.
[4] K. Nealson, W. A. Ghiorse, and E. Strauss, “Geobiology: Exploring the Interface Between the Biosphere and the Geosphere,” report based on a colloquium sponsored by the American Academy of Microbiology held December 1–3, 2000, in Tucson, Arizona (American Society for Microbiology, 2001). https://www.ncbi.nlm.nih.gov/books/NBK559441/.
[5] Vladimir Vernadsky, Geochemistry and the Biosphere: Essays (Synergetic Press, 2007).
[6] Christian S. Calude and Giuseppe Longo, “The Deluge of Spurious Correlations in Big Data,” Foundations of Science 22, no. 3 (2016): 592–612.
[7] Jennifer Gabrys, Program Earth: Environmental Sensing Technology and the Making of a Computational Planet (University of Minnesota Press, 2016).
[8] Benjamin Bratton, The Stack: On Software and Sovereignty (MIT Press, 2015).
[9] David J. Beerling and Nicholas J. Butterfield, “Plants and Animals as Geobiological Agents,” in Fundamentals of Geobiology, ed. A. H. Knoll, D. E. Canfield, and K. O. Konhauser (Blackwell Publishing Ltd., 2012).
[10] Beerling and Butterfield, “Plants and Animals,” 189.
[11] Salva Duran-Nebreda and George W. Bassel, “Bridging Scales in Plant Biology Using Network Science,” Trends in Plant Science 22, no. 12 (2017): 1001–03.
[12] R. Dale et al., “Overcoming the Challenges to Enhancing Experimental Plant Biology with Computational Modeling,” Frontiers in Plant Science 12. (2021). https://doi.org/10.3389/fpls.2021.687652.
[13] “Root-soil models are a good example of mechanistic mathematical models applied across scales (e.g., root branching and the biophysical processes involved in water uptake), as well as the seamless transition from the pattern modeling approach of morphology to mechanistic mathematical modeling”: R. Dale et al., “Overcoming the Challenges,” 5.
[14] A. Gorissen and A. J. C. de Visser, Modelling Carbon Sequestration: With a Focus on Grasslands, Effects of Climate and Management (Plant Research International, May 2004), https://edepot.wur.nl/31223.
[15] K. Nealson, W. A. Ghiorse, and E. Strauss, “Geobiology.”
[16] Donna Haraway, Staying with the Trouble: Making Kin in the Chthulucene (Duke University Press, 2016).
[17] Jeffrey T. Nealon, Plant Theory: Biopower and Vegetable Life (Stanford University Press, 2015).
[18] Eric D. Brenner et al., “Plant Neurobiology: An Integrated View of Plant Signaling,” Trends in Plant Science 11, no. 8 (2006): 413–19.
[19] Alfred North Whitehead, Science and the Modern World ([1925] Free Press, 1967), 51.
[20] Hesham S. Ghazzawy et al., “Paulownia Trees as a Sustainable Solution for CO2 Mitigation: Assessing Progress Toward 2050 Climate Goals,” Frontiers in Environmental Science 12 (2024): 1360–85.
[21] B. Marana, “A Green GIS Solution Against Air Pollution in the Province of Bergamo: The Paulownia Tree,” Journal of Geographical Information Systems 10 (2018): 193–218.
[22] Ghazzawy et al., “Paulowina Trees.”
[23] Giuseppe Longo and Maël Montévil, Perspectives on Organisms: Biological Time, Symmetries and Singularities (Springer, 2014) and F. Bailly and G. Longo, Mathematics and the Natural Sciences: The Physical Singularity of Life (Imperial College Press, 2011).
[24] P. C. W. Davies, “The Epigenome and Top-Down Causation,” Interface Focus 2, no. 1 (2012): 242–48 and Giuseppe Longo and Maël Montévil, Perspectives on Organisms.
[25] Cited in Joseph Needham, “A Biologist’s View of Whitehead’s Philosophy,” in The Philosophy of Alfred North Whitehead, ed. Paul Arthur Schlipp (Tudor Publishing Company, 1951), 254.